In this exercise you will be studying event displays from the ALEPH detector at the CERN lab in Geneva, Switzerland. You'll use your powers of deduction to try and measure an important prediction of the Standard Model: the ratio of two types of Z0-boson decay.
LEP and the Z0 boson
ALEPH was an experiment on the LEP (the Large Electron-Positron collider), which ran until 2001. The 27 km tunnel that housed the LEP collider now contains the Large Hadron Collider (LHC):
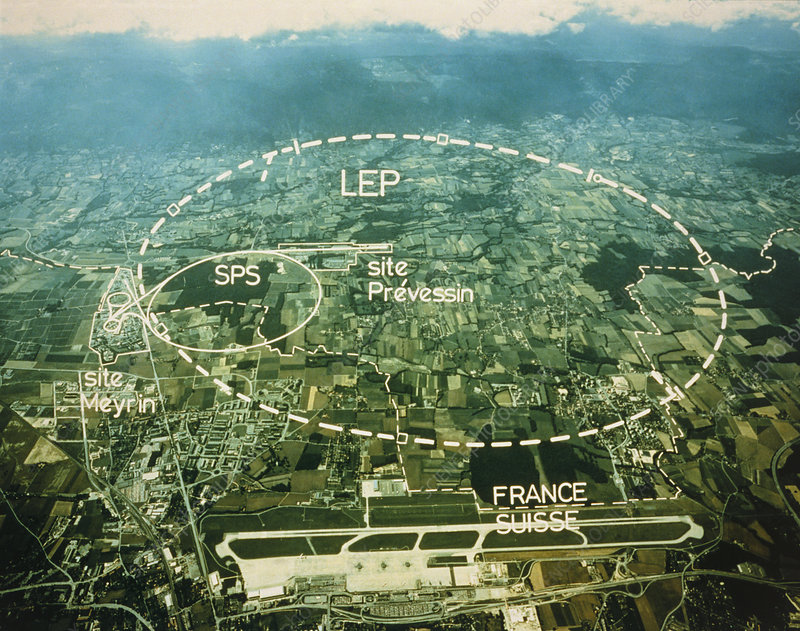
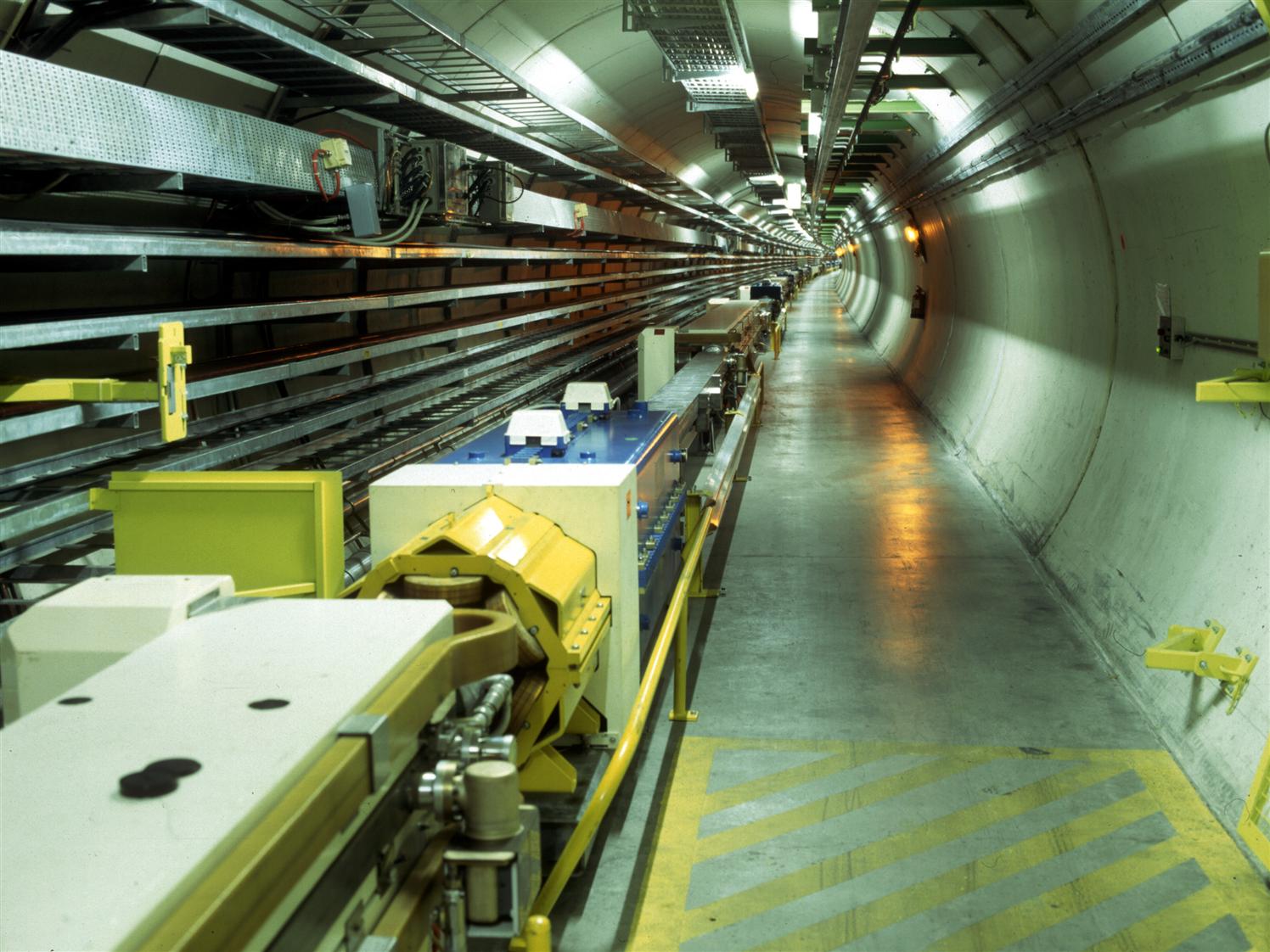
LEP's main legacy is precision studies of the Z0 particle, the electrically neutral carrier of the weak force. The Z0 can decay in several ways, but we will focus on its decay to fermions, "matter" particles which come in two groupings: leptons and quarks. We are going to measure the relative rates of these decays: as the Standard Model (SM) specifies how many types of lepton and quark are available to decay into and how strongly they interact, we can use the decay rates to test the SM.
You might wonder why we take the ratio rather than just using the lepton and quark rates directly. Ratios are very experimentally useful, as they cancel out various systematic uncertainties, e.g. the shape of the detector, which apply to both parts, so the ratio can be measured more precisely than its components.
The ALEPH detector
The electrons and positrons (anti-electrons) accelerated by LEP are made to collide, with a centre-of-mass energy matching the 91 GeV mass of the Z0 boson, at several interaction points around the ring. At these points, the colliding beams are surrounded by complex particle-detector apparatus, one of which is the ALEPH experiment. A cutaway drawing of the ALEPH detector is shown here:
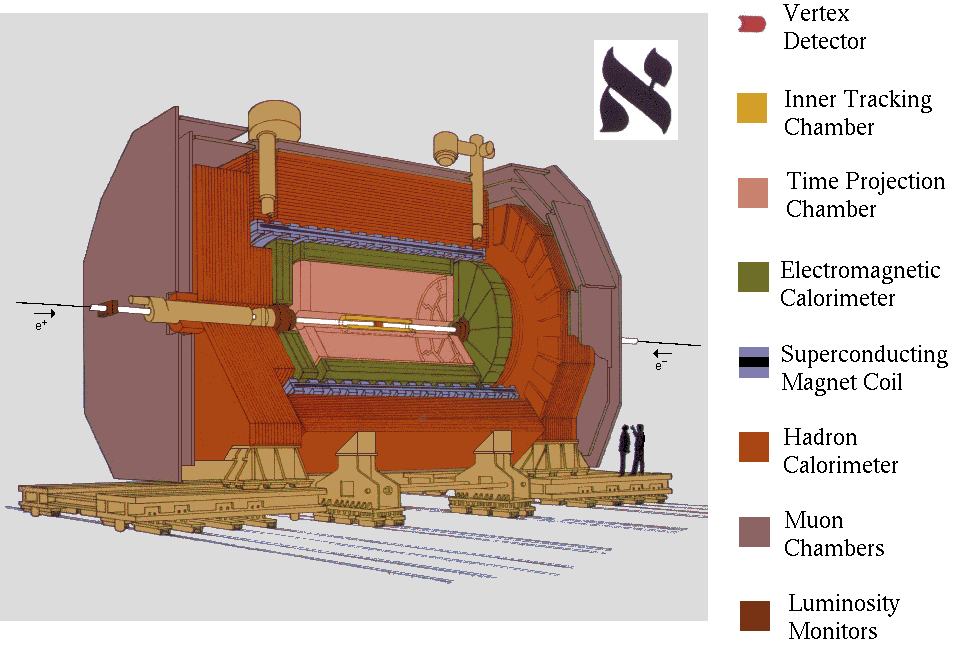
Beams of electrons and positrons enter the detector as shown by the arrows. They collide, creating Z0's in the middle of the detector. The Z0's decay almost instantly (10-24 seconds), and the leptons or hadrons into which they decay travel through the detector.
ALEPH is composed of cylinders of different types of particle detection technology, wrapped around the beam-line, as shown in the following image:
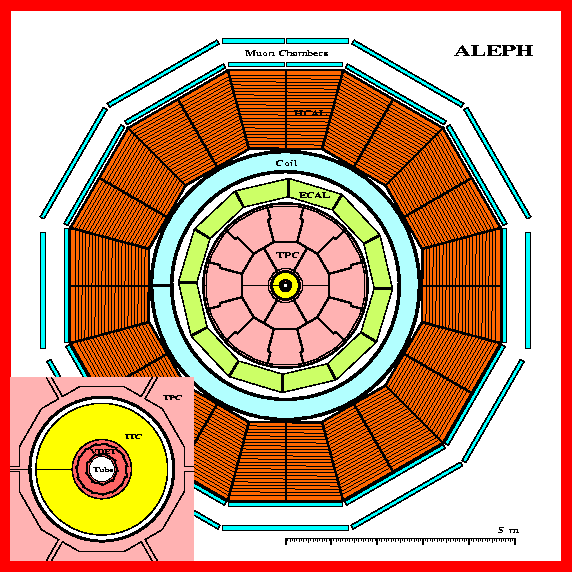
Starting from the centre, the most important types of particle detector are
- Tracker:
- the tracker is made of thin material that responds to transit of charged particles. This detector obstructs the particles as little as possible, but is very good at measuring their positions. The tracker is contained in a magnetic field, so the charged particles follow curved paths: the radius of curvature lets us estimate the particles' momenta.
- ECAL:
- the electromagnetic calorimeter, or ECAL, is a detector composed of layers of lead and detector electronics that is very good at make electrons (and positrons) and photons deposit all their energy. It is not so precise at position, but very good at energy measurements. Hadrons and muons deposit a little energy in the ECAL, but much less than electrons and photons.
- HCAL:
- the hadronic calorimeter, HCAL, is a sandwich of steel and readout detectors. It is similar to the ECAL, but designed to absorb the energy of hadrons rather than electrons and photons (which should nearly all be stopped by the ECAL).
- Muon system:
- Particles that still survive the trip beyond HCAL are muons and they will leave a track in the hadron calorimeter as well as go on to the muon chambers, two layers of Geiger-like tubes that surround the rest of ALEPH.
Identifying particles
The different responses of different particle types give us a way to identify the mix of particles in the final state of the collisions:
- Photons:
- photons are particles of light, and have no charge. So they will not leave tracks in the inner detector, but dump all their energy into the ECAL.
- Electrons:
- electrons are charged and so leave tracks. They also shower profusely and usually lose all their energy in the ECAL, not reaching the HCAL or muon system.
- Muons:
- muons are like heavy electrons, so they are charged and leave tracks. However, their extra mass means they don't interact much in the calorimeters (they are "minimum ionizing" particles) and hence get all the way through to the muon system. Despite being unstable particles, at the energies involved in Z0 decays, muons do not usually decay within the detector.
- Neutrinos:
- the uncharged cousins of the electron, muon, and tau, neutrinos come in all three lepton flavours, and hardly interact at all: they can happily pass through the earth, and specialist experiments build huge underground detectors to visualise just a tiny fraction of them. At colliders, neutrinos are visible only as "missing energy": as the initial particles have no momentum perpendicular to the beamline, if you add up all the visible particles' momentum vectors and get a non-zero momentum, that indicates that something invisible – usually a neutrino – was also produced.
- Taus:
- tau leptons are super heavy electrons, and decay much faster than muons: they most likely do not personally hit the detector at all, but rather their decay products do. And they can have many decay modes: taus can decay to either electrons or muons (producing two unseen neutrinos in the process, or to hadrons via quarks (and one neutrino). Discriminating taus from either electrons/muons or hadronic jets can be difficult, with a major signature being lower energies in the visible particles, and non-balancing momenta perpendicular to the beamline — both features because the neutrinos take unseen momentum away.
- Hadrons/jets:
- quarks and gluons are not happy to be seen "naked", and as they move away from each other they eventually pop new quark-antiquark pairs into existence. This "shower" of particles eventually resolves itself into a jet of hadrons. Charged hadrons will leave tracks, neutral ones will not; charged ones will also leave some energy in the HCAL; but both charged and uncharged will be caught by the HCAL so the hadrons will not reach the muon system... unless some heavy hadron decays to a muon in the process. Directly produced jets contain a lot of particles compared to the other modes: even hadronic tau decays are typically only to a few (and odd-numbered) tracks.
Identifying Z0 decays
The Z0 boson will always (in this exercise) decay to a pair of fermions, either leptons or quarks. Running at the LEP1 resonance mass, the Z0 will be stationary, and so the decay must be back-to-back with one decay product going in the exact opposite direction to the other, to balance the momentum. When identifying event types, look for these two opposing flows of particles, lighting up the detector in their characteristic ways.
Z0 to an electron-positron pair
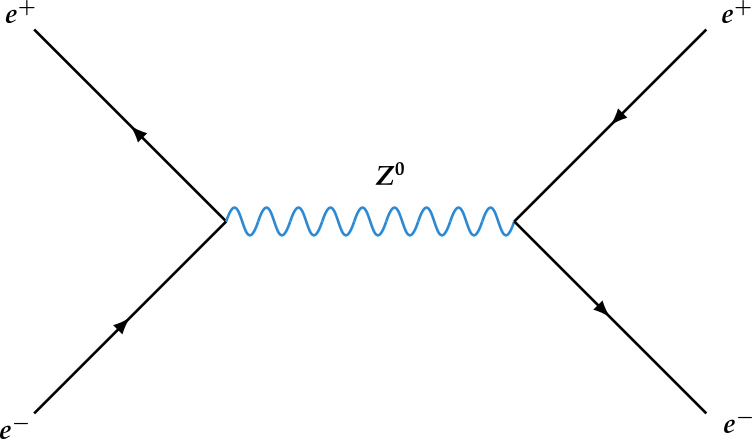
Note only two particles (no jets), tracks, nothing beyond the ECAL.
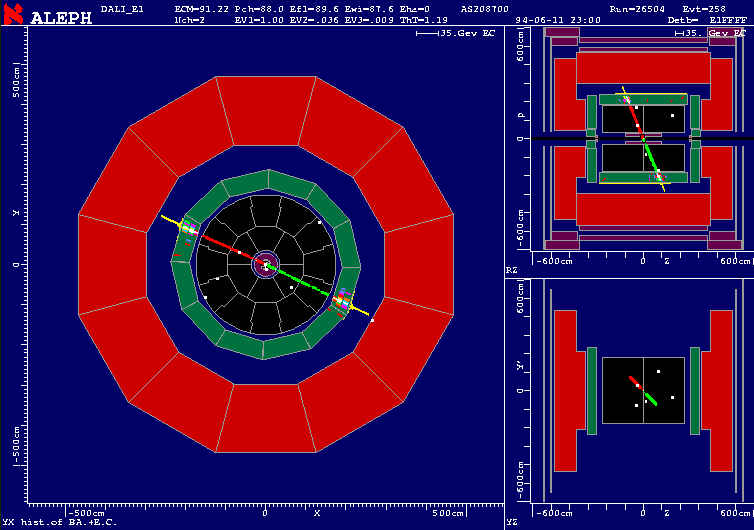
Z0 to a muon (μ-) and antimuon (μ+) pair
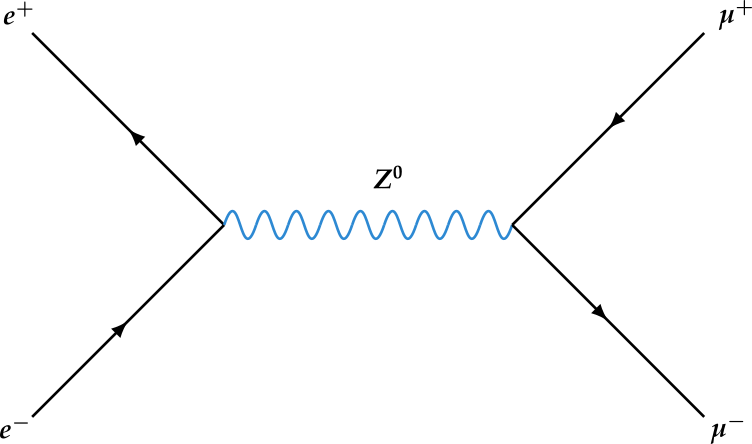
The idea is similar: just two tracks, now minimal interactions in the calorimeters, and activation of the outer muon system.
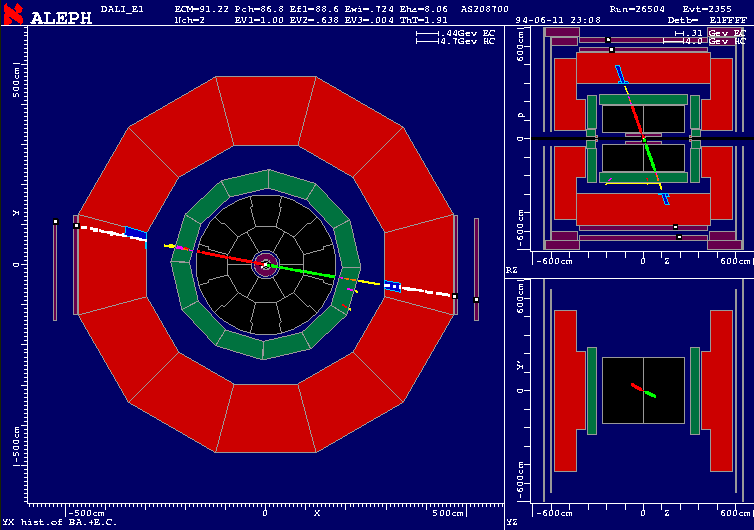
Z0 decays into hadrons
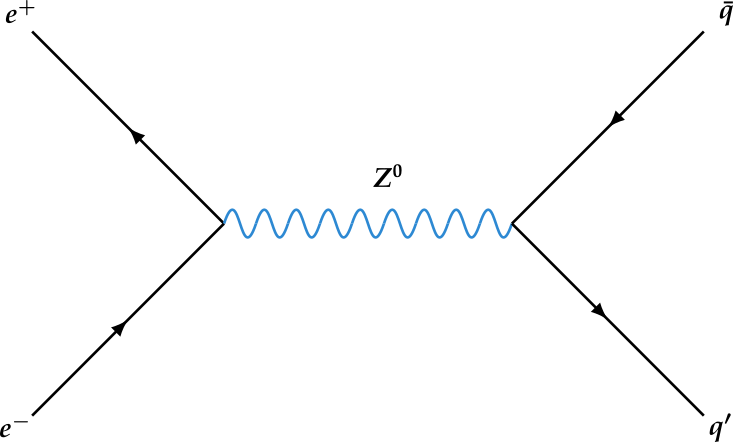
The hadronic decays of the Z0 are really decays into quark-antiquark pairs of one of the five following types of quark: u,d,s,c or b. The top quark is not involved because it is more massive than the Z0.
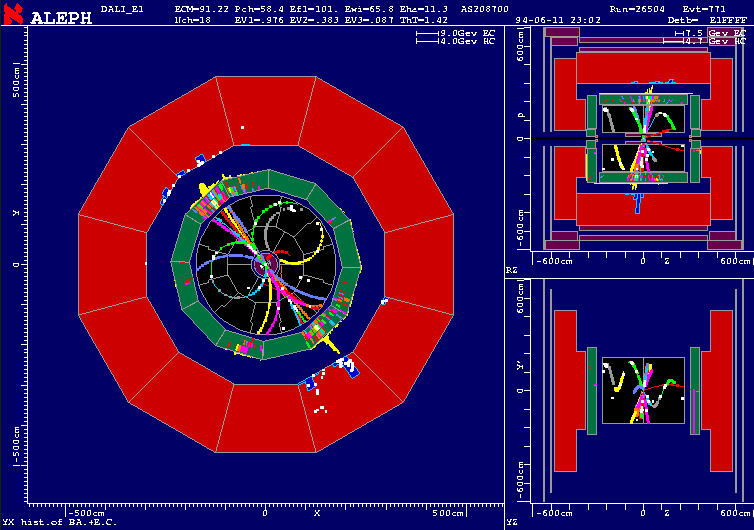
Z0 to a tau and anti-tau pair
We've left taus until last: they are usually harder to identify than the event-types above, because taus fake the other particles by rapidly decaying into them!
The physics behind this is that each tau itself into a tau neutrino and the charged carrier of the weak force, the W+ or W- boson. The W then decays into quarks or into two leptons, one of which is a neutrino. An example of this is shown here for the case where both taus decay into electrons:
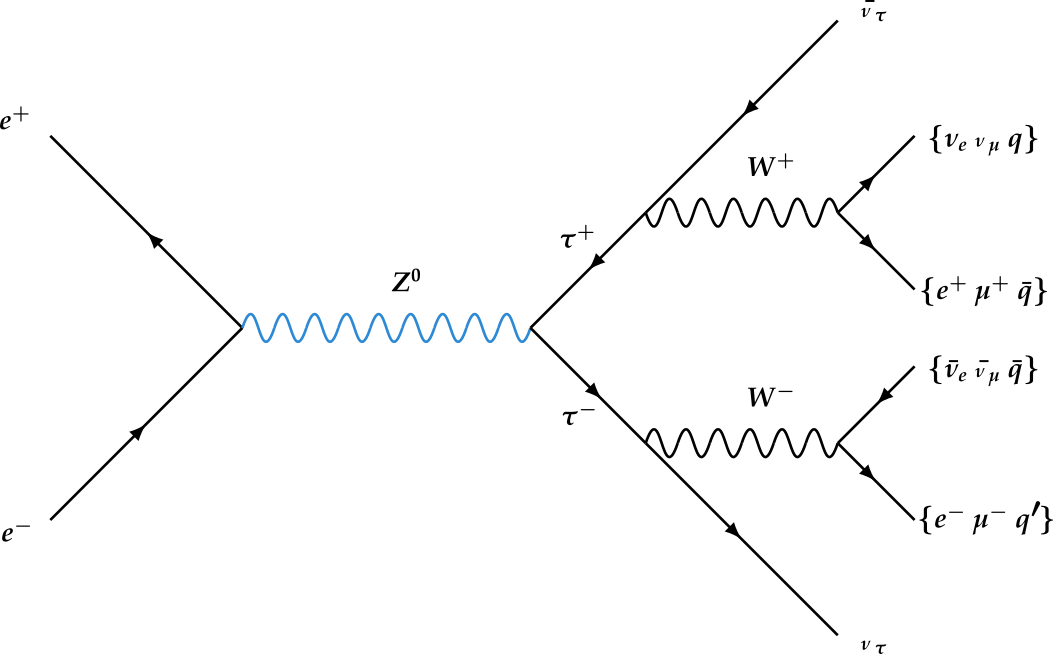
This looks quite complicated, but if one just studies what is coming out of the center of the detector, lumping all the neutrinos into one missing energy arrow, it looks more like these:
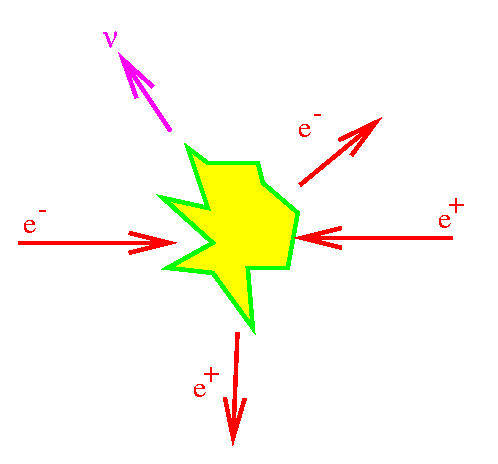
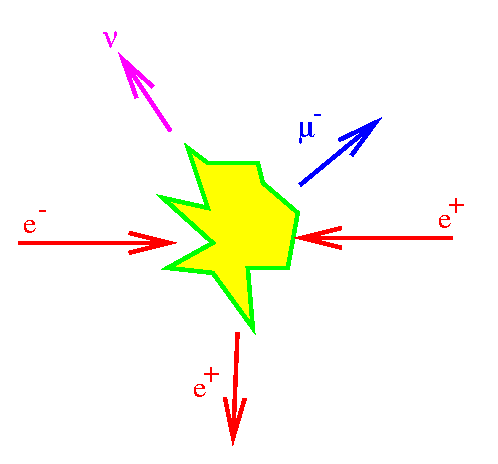
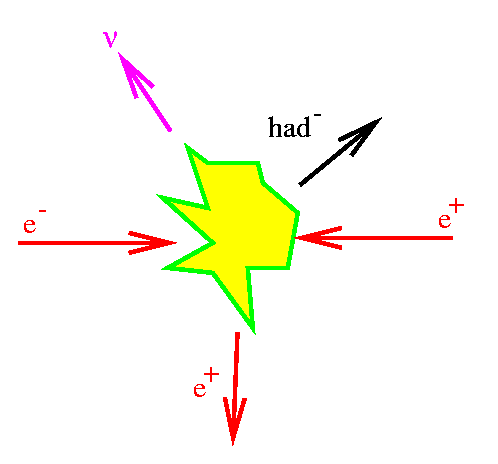
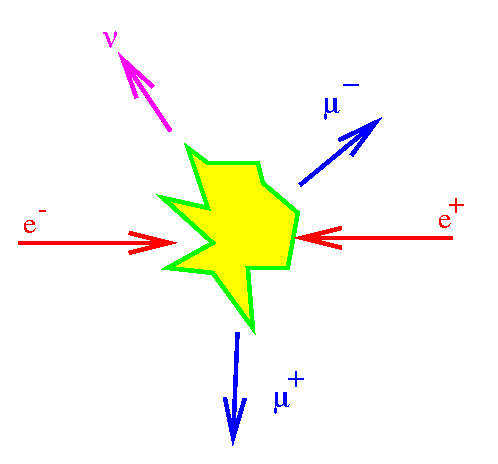
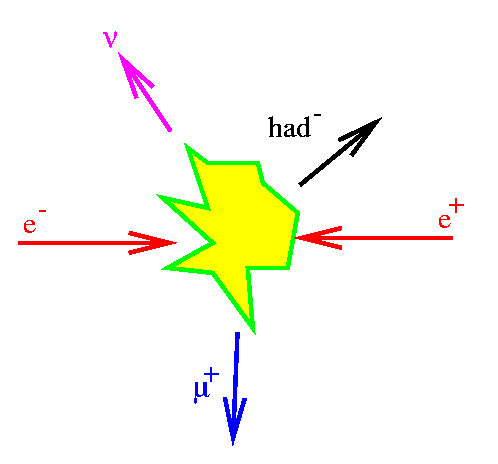
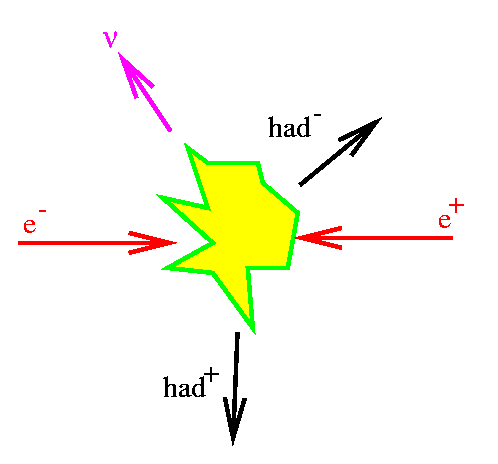
Remember there are two taus in the event, and they decay independently, so we can end up with all the combinations: electron/electron, electron/muon, electron/hadron, muon/muon, muon/hadron, and hadron/hadron.
The most distinctive features of tau events are i) asymmetric decays, i.e. different particle types on each side of the detector, and ii) energy imbalance, i.e. the resulting particles are not back-to-back due to missing energy through the invisible neutrinos.
Tau events are sneaky: if you get a symmetric tau decay into e-e, mu-mu, or quark-antiquark combinations, they will look like those event types unless there's some clear missing energy (which can also be faked by an imperfect detector). And if the two neutrinos' missing momentum cancel each other out, it can be nearly impossible to distinguish a tau-tau from a "prompt" version of the same final state! You will probably find tau events hardest to identify.
Measuring R decays
Our ultimate aim is to measure the relative amounts of decay to the different fermions. An important summary number for this is R, the ratio of hadronic (quarks) to lepton (electron, muon, or tau) decays: $$ R = \frac{ \sigma(e^+ e^- \rightarrow \mathrm{hadrons})}{\sigma(e^+ e^- \rightarrow \ell^+ \ell^-) }, $$ where the \(\sigma\)'s represent the rates of production and the curly-\( \ell \) symbols represent the leptons. R is a prediction of the Standard Model, as it is sensitive to the quark electric charges, the prediction that there are three different colours of quark, and that there should be five active flavours of quark in this decay: the 173 GeV top quark is too heavy for the 91 GeV Z0 boson to decay to it. To a lesser degree, R is a test of how the masses of the different particles affect the decay rates. The accepted experimental value of the ratio, averaged across all three types of lepton, is \( R = 20.767 \pm 0.025 \). Let's see how close you can get!